/ SNI INSight
Entanglement: A fascinating topic of research in the SNI network – and worthy of a Nobel Prize
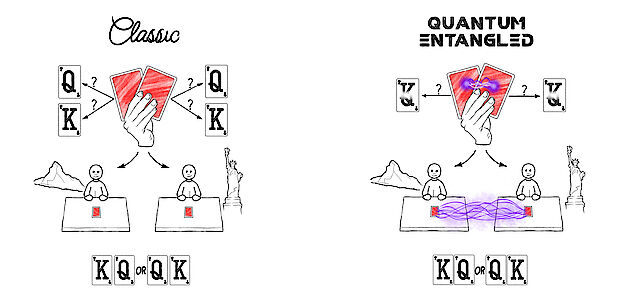
To illustrate the complex quantum world and entanglement, let’s imagine that two people living far away from each other each gets a playing card (namely a queen or king) and inspects it at the same time. In the classical world, one of the persons gets a queen, and the other gets a king. It is clear who obtained which card when the cards were distributed. In the quantum world, however, when the playing cards are sent out, they can be both queen and king at the same time – a quantum mechanical superposition. Only when these “magic” cards are revealed, it is decided by chance whether it is a queen or a king. If the magic cards were entangled before being distributed, there is a close connection between them that remains – even over a long distance. If one person reveals a queen, the other person always possesses a king – and that, although for both cards it is decided whether they are queen or king only at the moment when the cards are turned face up and they both have the potential to be queen or king. (Image: SNI and Scixel)
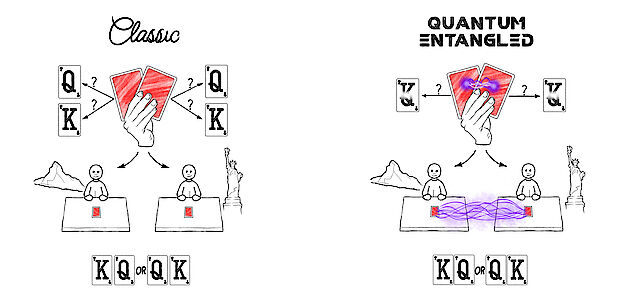
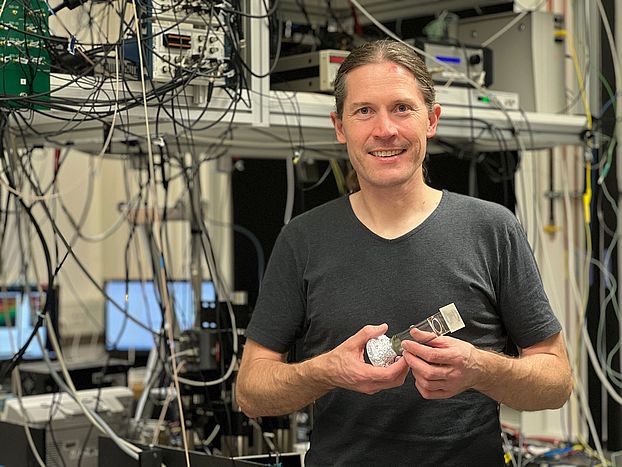
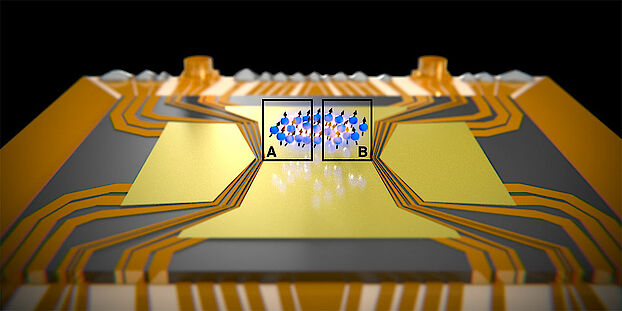
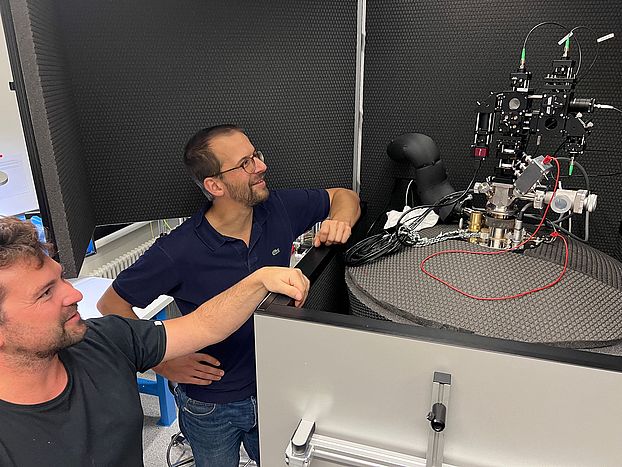
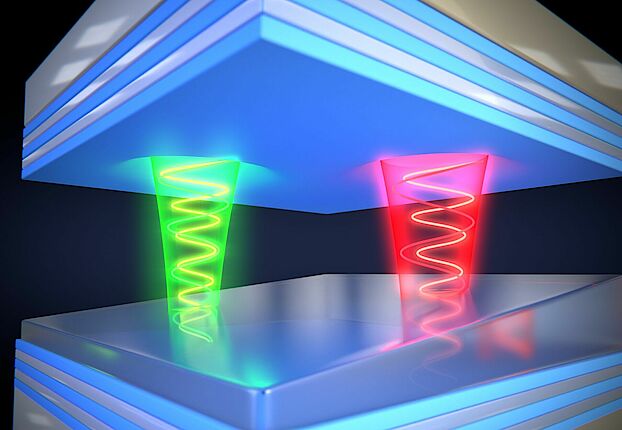
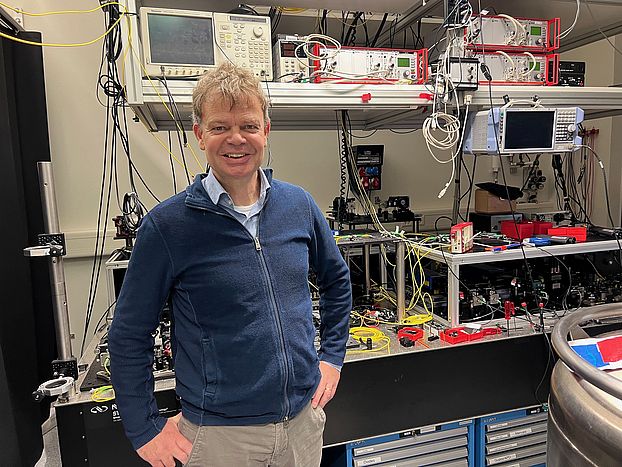
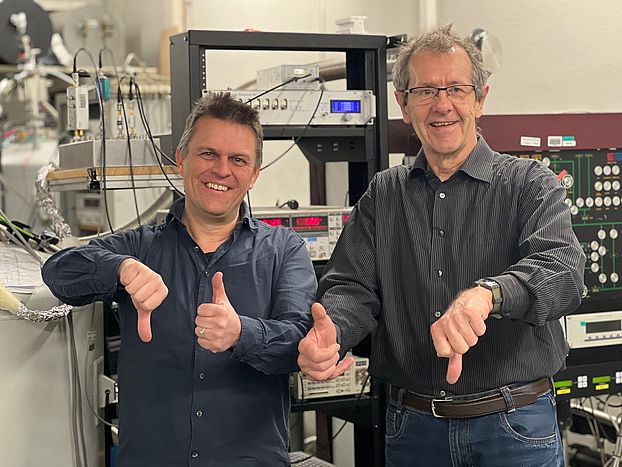
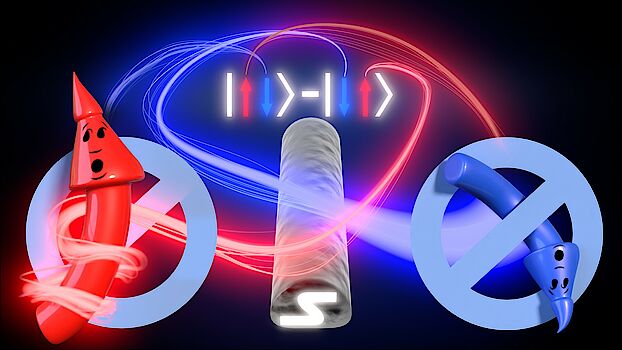
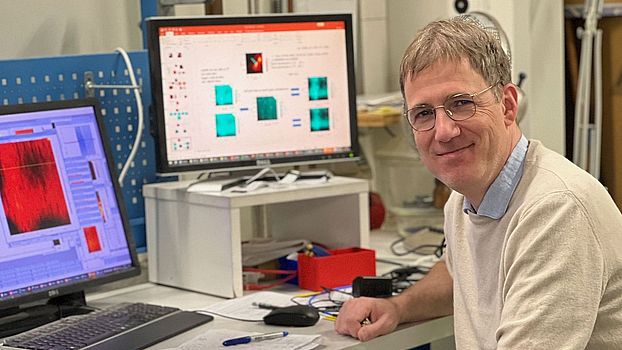
In October, the Nobel Prize Committee announced that Professors Alain Aspect, John Clauser and Anton Zeilinger were to be awarded this year’s Nobel Prize in Physics. Their pioneering experiments with entangled photons have laid the foundations for fundamental physical experiments and various quantum applications of quantum mechanics. Within the SNI network, various research groups have also been working on experimental investigations of entanglement and have set their sights on specific applications. Here, we look at several examples that illustrate how researchers at the Department of Physics of the University of Basel are also investigating this quantum phenomenon, which is so hard to reconcile with our experiences of the macro world.
A close connection even over long distances
Entanglement is a quantum physical phenomenon in which certain properties of particles are closely connected with one another, even if the particles are separated by thousands of kilometers. The relationship between them remains intact, provided they are not disturbed. The entangled properties can involve the wavelengths of photons or the orientation of magnetic moments (spins) of particles.
The fact that a connection like this works without some kind of communication going on in the background is impossible to reconcile with our experiences of the macro world. Albert Einstein is among the researchers who have studied the phenomenon of entanglement — and rejected the idea that a strong correlation between two particles could exist in this way. He described the phenomenon as “spooky action at a distance” and assumed that it must be underpinned by hitherto unknown classical variables.
Correlations demonstrated experimentally
In experiments, however, the three Nobel laureates have demonstrated that this entanglement actually exists — at least in the quantum and nano world. The basis for their analyses are so-called Bell tests, in which researchers initially assume that particle properties exist independently of one another and at a specific location. A measurement of one particle should not therefore have any influence on that of another — in other words, one revealed card should be independent of the other (see image 1) — as we’re familiar with in the macro world. Based on these considerations, it’s possible to derive an inequality that the measurement results should fulfil.
Yet the Nobel laureates’ experiments with entangled photons that were sent to spatially separate measuring stations showed that it is possible for this “Bell inequality” to be violated. The properties of the photons in the experiments depend on one another — in other words, there is a “Bell correlation” between them. The particles don’t assume their properties until a measurement is taken, contradicting our experiences in the macro world — after all, if we cast our minds back to our thought experiment in the macro world, the card was already a queen or a king before it was revealed.
These fascinating phenomena are also the subject of research by several research groups at the Department of Physics at Basel University, which forms part of the SNI network. The various teams pursue very different strategies when it comes to creating and investigating entangled particles — and are also pursuing different objectives in their research. Common to all of the groups is a curiosity about the laws of the quantum world and an interest in understanding these laws in order to pave the way for future applications.
Does quantum mechanics stop working at some point?
Like the three Nobel laureates, Professor Philipp Treutlein and his team also study quantum mechanical phenomena in the context of basic science — albeit not using individual photons but rather many-particle systems containing more than a thousand atoms.
“The fundamental question behind our research is whether these quantum mechanical phenomena are subject to a fundamental size limit, which ensures that the macro world obeys the classical laws we’re familiar with in our everyday lives — after all quantum phenomena generally disappear in large systems. Alternatively, do the quantum laws that include phenomena such as entanglement also apply in the macro world, and is detecting them simply a question of effort and the techniques we use,” explains Treutlein. “To explore this question, we’re creating entanglement and Bell correlations in increasingly large systems with an ever-greater number of particles.”
Experiments with ultracold atom clouds
In their experiments, the researchers use laser light to cool a cloud of ultracold atoms to temperatures just a few billionths of a degree above absolute zero. Inside this cloud, collisions are constantly taking place between atoms — which in turn cause the magnetic moments (spins) of all of the atoms to become entangled with one another. “If we then take any two atoms in the cloud, they form an entangled pair — in other words, if the spin of one atom is pointing upwards, the other is automatically pointing downwards,” explains Treutlein, although the direction is not defined until the magnetic moment is measured. Again, this is another of the unusual features of the quantum world.
In this cloud of ultracold, entangled atoms, the researchers can then study areas that are spatially separated from one another. To do so, they measure the correlation of spins in the separated areas and determine the precise position of the atoms. Based on the measurements in one specific area, Treutlein’s team can then also predict the results in another area. “We recently managed to split the cloud and spatially separate the two parts,” says Treutlein. “Here, too, measurements can prove that the spins of the two clouds are entangled with one another. Measuring the spin in one cloud influences the result of the measurement in the other — just as in the ‘spooky action at a distance’ that Einstein described.”
Reduction of quantum noise
Through these experiments, the researchers demonstrate not only that the laws of quantum mechanics apply to the many-particle system in question, but also that these laws could potentially pave the way for numerous applications.
Potential applications include more accurate sensors and imaging techniques. Interestingly, entanglement can be used to determine — and even modify — the state of one particle by measuring the other. Treutlein’s team is using this phenomenon to improve the measurement accuracy of devices known as atom interferometers, which are some of the most precise instruments for measuring gravitation, electromagnetic fields and other fundamental quantities.
In measuring an electromagnetic field, an atom interferometer takes the mean of the measurement results for a large number of atoms. Since the measurements of individual atoms are random in the quantum world, however, there are fluctuations in the measured values. Although these fluctuations are reduced by averaging the measurements, some “quantum noise” always remains. In the case of entanglement, however, the particles are no longer independent of one another and instead behave like a unit. The quantum noise of the individual atoms is correlated, and the measurement precision can be significantly improved by skillful selection of the measuring technique.
Greater security in communication
“Theoretically, entanglement could also be used to improve security in communication,” explains Treutlein. This would involve encrypting the information to be exchanged using a key that was based on entangled quantum systems — generally photons. If someone intercepted this key, the sender and recipient would be able to tell immediately based measurement results of the partner photons. If no one had broken into the system, however, the message could be encrypted and sent.
Entanglement for better sensors
Professor Patrick Maletinsky also sees benefits in using entanglement when it comes to his research objectives. As well as developing sensitive sensors for tiny magnetic and electric fields, he is also working on quantum communication in collaboration with Professor Richard Warburton.
“Our research uses nitrogen-vacancy centers in diamonds. Within these vacancy centers, the spins of individual orbiting electrons change when the particles are exposed to an electric or magnetic field. The electrons can be excited and then emit individual photons that provide information about their spin state. This technique provides us with information on changes in individual spins based on the examined fields,” says Maletinsky, explaining the principle underlying his research.
Maletinsky and his team are searching for ways to make measurements even more accurate by increasing the number of sensors and taking an average of the results — as with atoms in the atom interferometer. To obtain a measurement that is 10 times more accurate, he must increase the number of sensors from one to 100. If the spins of the sensors were all entangled with one another, however, a tenfold increase in the number of sensors would theoretically be sufficient to obtain 10 times the measurement accuracy. “At the moment, the problem with our experiments is that it still takes us far too long to entangle the spins and that we then have too little time to take the measurement. We need to solve this issue before we can make practical use of entanglement in our sensors,” says Maletinsky.
Mirror systems for better communication
In collaboration with Warburton’s team, the group at Patrick Maletinsky’s Quantum Sensing Lab also wants to apply the entanglement of electrons to diamond sensors for quantum communication.
To produce entangled spins, the researchers use tiny depressions, known as cavities, in which two parallel mirrors ensure that a photon is not lost but is instead reflected back and forth and can interact with quantum systems in between. In a cavity of this kind, a photon can couple two quantum systems, such as electrons, with one another and entangle their magnetic moments (spins). Another possibility is to equip multiple cavities with their own quantum systems. In each cavity, the photon can be entangled with the spin of another particle, such as an electron. A suitable experimental setup can cause the photons in the various cavities to interfere with one another, leading to “remote entanglement” between the separate spins. The researchers are still working on implementing this kind of entanglement within their system with a view to further increasing the efficiency of their approach.
Quantum dots as a source of quantum light
In addition to his collaboration with Patrick Maletinsky on nitrogen-vacancy centers, Richard Warburton also works on approaches involving quantum dots — tiny nanoscale structures in semiconductor materials in which “trapped” electrons can adopt only very specific energy levels. An electron can be promoted from the lowest energy level to a higher one with a laser pulse. A single photon is created when the electron drops back down again.
Recently, the Warburton team has succeeded in using this method to produce almost completely identical photons from two separate quantum dots. If these photons then strike a semi-transparent mirror, they become entangled and always react as a pair — as seen in the phenomenon of remote entanglement described above. The photons then either pass through the mirror together or are both reflected. In the macro world, which we are much more familiar with, it would also be possible for one of the two photons to be reflected and the other to pass through the mirror, but this is not the case with identical photons.
Aiming for cluster and graph states
“Above all, we’re working on producing not only an entangled photon pair but rather chains of entangled photons called cluster states, subsequently also more complex graph states with entangled photons,” says Richard Warburton. “These larger structures with multiple entangled photons could be used in quantum communication or even in a quantum computer.”
Although the principle used to produce these larger entangled structures doesn’t sound all that complicated in theory, it is a challenge to produce more than one entangled photon pair in practice. In their approach, the researchers use a quantum dot with a single electron. They excite this electron so that it emits a single photon, which becomes entangled with the electron’s spin. Given that light moves very quickly, the photon disappears immediately after it is emitted — but its entanglement with the electron persists. The electron is then excited again, producing a second photon that becomes entangled with the first photon. “After the process is run for a third time, a short chain of three entangled photons can be formed. We could then extend this chain even further — and although the photons are at different locations, the close connection between them would persist,” says Warburton, describing his team’s approach. “Creating a graph state of entangled photons is a long way off, however.”
Entangled pairs for free
Whereas it’s very expensive for some lines of research to entangle photons or electrons with one another, the team led by Professor Christian Schönenberger and Dr. Andreas Baumgartner receives entangled electron pairs “for free.” As electrons in superconductors are always entangled in pairs, the researchers from the Quantum- and Nanoelectronics group use superconductors as a natural source.
Some time ago, the team developed an electronic device component that can be used to extract such electron pairs, known as Cooper pairs, and then separate them spatially. This separation takes place in two spatially separate traps, known as quantum dots, in which the electrons are captured for a short time.
Only recently have the researchers then been able to demonstrate a negative correlation between the spins of the two separated partners of a pair — in other words, one partner is always “spin up,” and the other “spin down.” The researchers achieve this using two spin filters that can be configured to allow electrons with a specific spin orientation to pass. For example, if both spin filters are configured so that only spin up electrons can pass, the flow of current is suppressed. If the two spin filters are pointing in opposite directions, however, an electric current flows.
“The electrons in the Cooper pairs are not separated from one another by a sufficient distance, however, and the experiment can therefore not be considered as a Bell test with electrons — but we’re working on it,” says Andreas Baumgartner.
“Entangled electrons of this kind could be used in the realization of a quantum computer,” explains Christian Schönenberger. “For example, a superconductor could be placed between two storage units (qubits) of the quantum computer to connect them via entangled Cooper pairs. Until now, the necessary interactions between two qubits only worked if they were very close to one another — which isn’t ideal when it comes to implementing a larger system.”
Elementary for quantum computing
For the development of a spin-based quantum computer, to whose development Professor Dominik Zumbühl’s group is contributing, the entanglement of multiple spins is a basic requirement.
A quantum computer does not work with bits like a conventional computer, but with quantum mechanical states of suitable systems, so-called qubits. Various possibilities for the realization of qubits are being discussed worldwide. Researchers in Basel follow the approach to realize a qubit by the magnetic moment (spin) of an electron. Unlike a bit in a conventional computer, the spin of an electron can not only assume two states (0 and 1), but can point up, down and in numerous other directions simultaneously. These different states of the spin are superimposed and are not fixed until they are measured – leading to the much higher computing power of a quantum computer compared to a conventional computer.
The Zumbühl team is investigating methods to realize such a spin-based computer in semiconductors. The difficulty here is to keep the electron spin stable and to control it. The researchers are already quite successful to control individual electrons by applying electric fields. In addition, however, several spins have to be coupled with each other – which in the quantum world is done by entanglement.
Researchers from Dominik Zumbühl’s Quantum Coherence Lab recently succeeded in producing two qubits whose spins can be controlled arbitrarily and which are entangled with each other. “The two quantum systems are then no longer independent of each other, but react as a unit,” explains Dominik Zumbühl. “With two qubits, this does not yet lead to a drastic acceleration in computing power. But if the number of qubits is increased, it makes a serious difference, since the computing operations can be executed in parallel rather than one after the other in the case of entangled qubits.”
Collaboration is key to success
The projects described here always involve whole teams of people — not only from the University of Basel but also from research institutions all over the world. Close collaboration with theoretical physicists is also hugely important. In Basel especially, with the groups led by Professors Christoph Bruder, Jelena Klinovaja, Daniel Loss and Patrick Potts, there are some excellent theoretical scientists who work closely with researchers in the field of experimental physics and provide impetus for novel applications.
The awarding of the Nobel Prize to the three quantum researchers Alain Aspect, John Clauser and Anton Zeilinger has certainly helped even more to focus public attention on quantum research. Although it is still difficult for non-experts to imagine how the phenomenon of entanglement between particles works, the examples set out here demonstrate that this quantum mechanical coupling is a fascinating area of research — and one that paves the way for numerous potential applications.
All of the above approaches differ from one another and help to improve our understanding of the laws of quantum mechanics, as well as helping researchers learn to overcome the enormous technical hurdles. Despite huge advances in quantum research in recent years, there are still many question marks and technical challenges when it comes to controlling individual particles and these tiny structures.
Further information:
Research group Philippe Treutlein
Research group Patrick Maletinsky
Research group Richard Warburton
Research group Christian Schönenberger
Research group Dominik Zumbühl